Master Thesis Prize 2023 – Honorable mention
Nuclear Magnetic Resonance (NMR) is a method used extensively for measuring the composition and structure of matter. One common use is Magnetic Resonance Imaging (MRI). At this point, we need a recap on NMR. It is like a two-state quantum mechanical tuning fork in which the resonance frequency of signal has been determined by a nucleus and magnetic field density of external field. Analogous to a tuning fork, we excite the NMR system using electromagnetic wave at resonance frequency. From a tuning fork, we get a sound wave as a response but in NMR, the response is the Free Induction Decay (FID). The resonance frequency is called Larmor frequency in NMR. FID signal is corrupted by noise which decreases the performance of NMR machines, characterized by Signal-to-Noise Ratio (SNR). When designing an MRI machine, we want the SNR to be as high as possible to get better image quality.
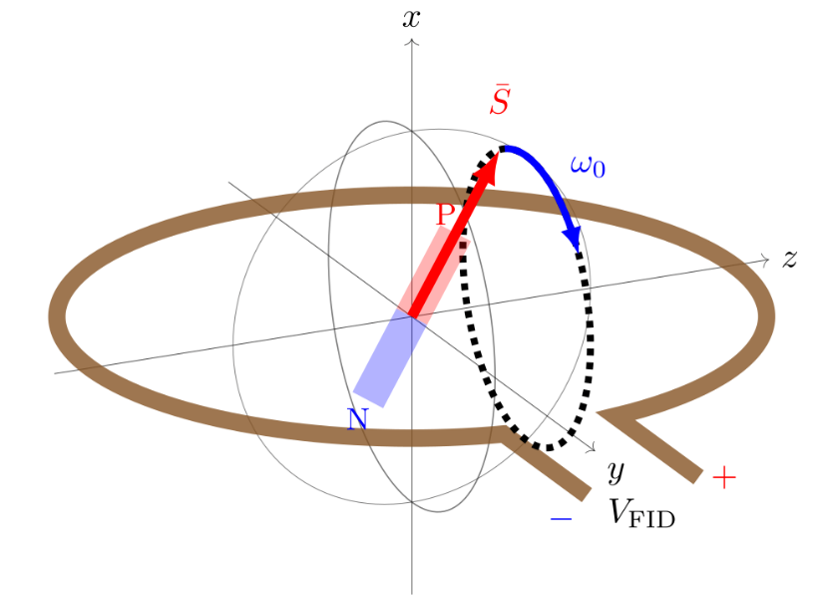
Figure 1: Generation of FID from nuclear precession. A nucleus with spin causes a net magnetization presented by a bar magnet. It rotates with angular frequency ω0. The magnetization induces a voltage VFID to a measurement probe which in this example, is a loop coil.
Formerly, NMR has usually been implemented using strong, superconducting magnets. The superconducting magnets have magnetic field density of several tesla corresponding to Larmor frequency of a few hundred megahertz since the Larmor frequency is linearly proportional to the magnetic field density. In addition, the maximum amplitude of the FID signal is proportional to the magnetic field density of the magnet, leading to higher SNR and good image quality for MRI machines. This is a great advantage in high-field MRI machines. However, this design has several drawbacks, for example, high cost and immobility. Current research, in addition to the ultra-high field, has shifted focus towards low-field NMR in which smaller and more affordable magnets can be used. One goal could be, for example, to design a low-field MRI machine small enough to fit in an ambulance. Low-field NMR machines have some drawbacks. They tend to have much smaller SNR compared to high-field NMR machines, which is a major limitation for image quality in MRI. To increase the SNR, we need an accurate models to predict it. The SNR depends on both amplitude of the FID and noise level of the measurement device. In our work, we developed a model which predicts the shape and amplitude of the measured signal and noise level of an NMR machine. In our work, we constructed a low-field NMR spectrometer which has Larmor frequency of 7 MHz. This resonance frequency requires magnetic field density of 0,1644 T which we can achieve using electromagnets, decreasing the overall cost.
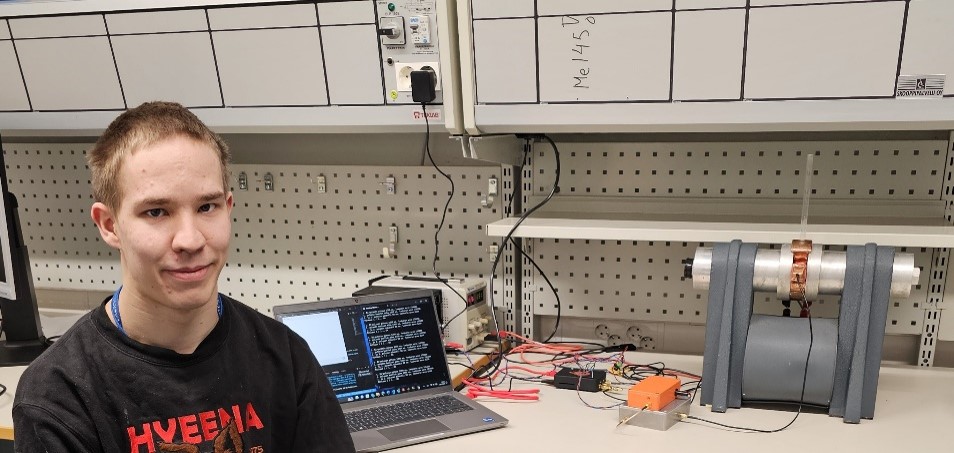
Figure 2: Self-made NMR spectrometer and the author of the thesis.
When designing low-field NMR devices, the noise level of the electronics has to be as low as possible. FID signal is very weak, at the scale of microvolts, so amplification is mandatory. Amplifiers for NMR are low-noise amplifiers designed for MRI, and this, as low noise as possible, is usually one of the most important factors in NMR devices. Another important factor affecting the overall noise of the device is the measurement probe itself. The measurement probe is a coil designed to receive FID signal as effectively as possible. To achieve good performance, optimization of the coil is required. There are several phenomena affecting the performance of the coil and design becomes more difficult due to the high frequencies. Due to the reasons above, it is challenging to design electronics for low-noise NMR machines. In this work, the coil is self-made, and it is based on previous work at similar Larmor frequency. However, better design of the coil may be possible by using numerical modelling of the coil which requires more research. Most low-noise amplifiers used in the device are commercial, but we also used some self-made amplifiers.
After the analog electronics, we process the amplified FID signal digitally to improve data quality. One approach is to use so called direct conversion which converts the measured FID signal to in-phase and quadrature phase data (IQ data). This conversion shifts the frequency content of a signal to zero frequency. Direct conversion is very common in radio receivers. It can be used to decrease noise level of the FID signal because it acts like a narrow band-pass filter. Many radio receivers have dedicated chip for direct conversion, which converts signal to IQ data before the analog-to-digital conversion. However, we can do it digitally after the analog-to-digital conversion. For this, we used a Field Programmable Gate Array (FPGA) which is a device that allows very fast and parallel digital processing of the FID signal. Final data is IQ data, which we use in several measurement programs.
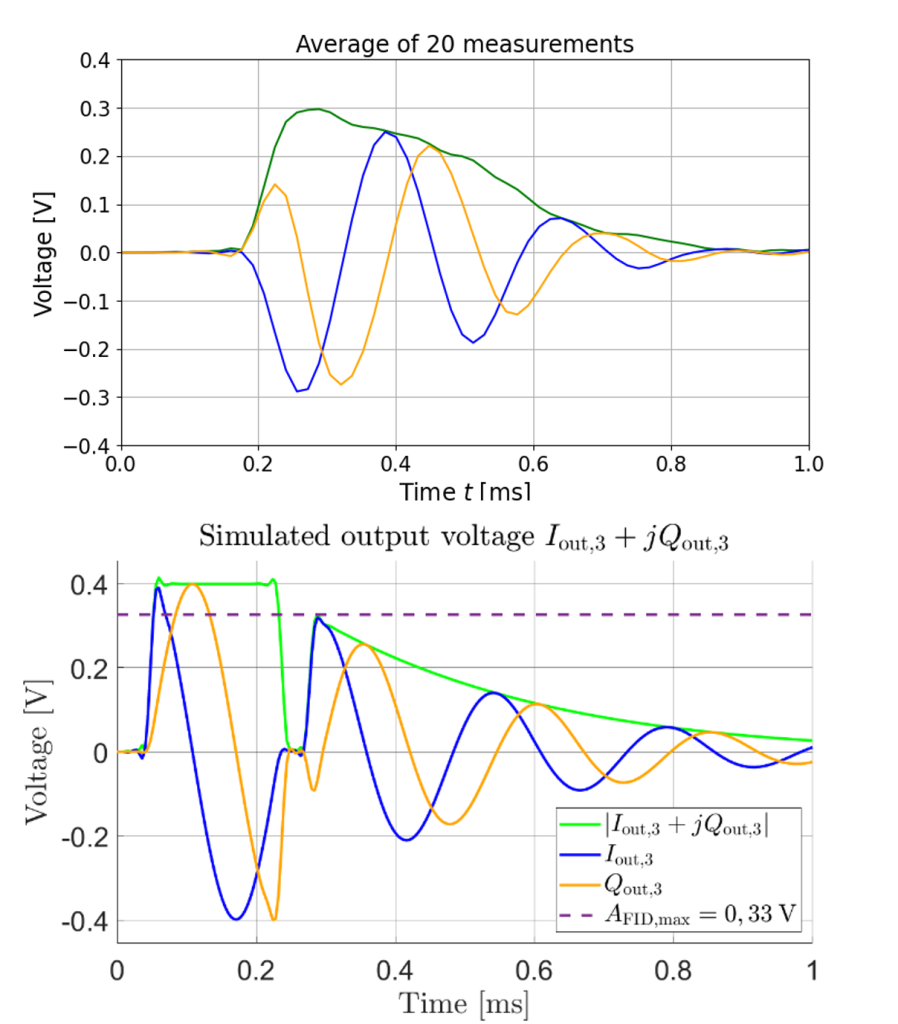
Figure 3: Measured and simulated FID signal compared.
We compared the output of simulation and measurements to verify our models. In both simulation and measurements, we used tap water as a sample. Our simulation is noise-free, so we ran it once. To reduce the noise level in the measurements, we averaged the measurements in time domain. As a result in both simulation and measurements, we received maximum amplitude of FID signal at 0,3 V. The simulated SNR is 71 and the measured SNR for single measurement is 13. Therefore, there is some unmodelled noise source in the simulation, which causes the difference. Our model also requires a set of nuclei at different magnetic field densities to enable more practical simulations. In the measurements, our FID signal should be a lot longer than 0,6 ms. This apparent short duration of the received signal is due to the electromagnet, which is not good enough for practical NMR spectroscopy, but it works well enough to give the FID signal. The NMR spectrometer built in this work can measure basic properties of a sample, but it should perform a lot better. In addition, next step would be to build a low-field MRI machine based on the spectrometer.